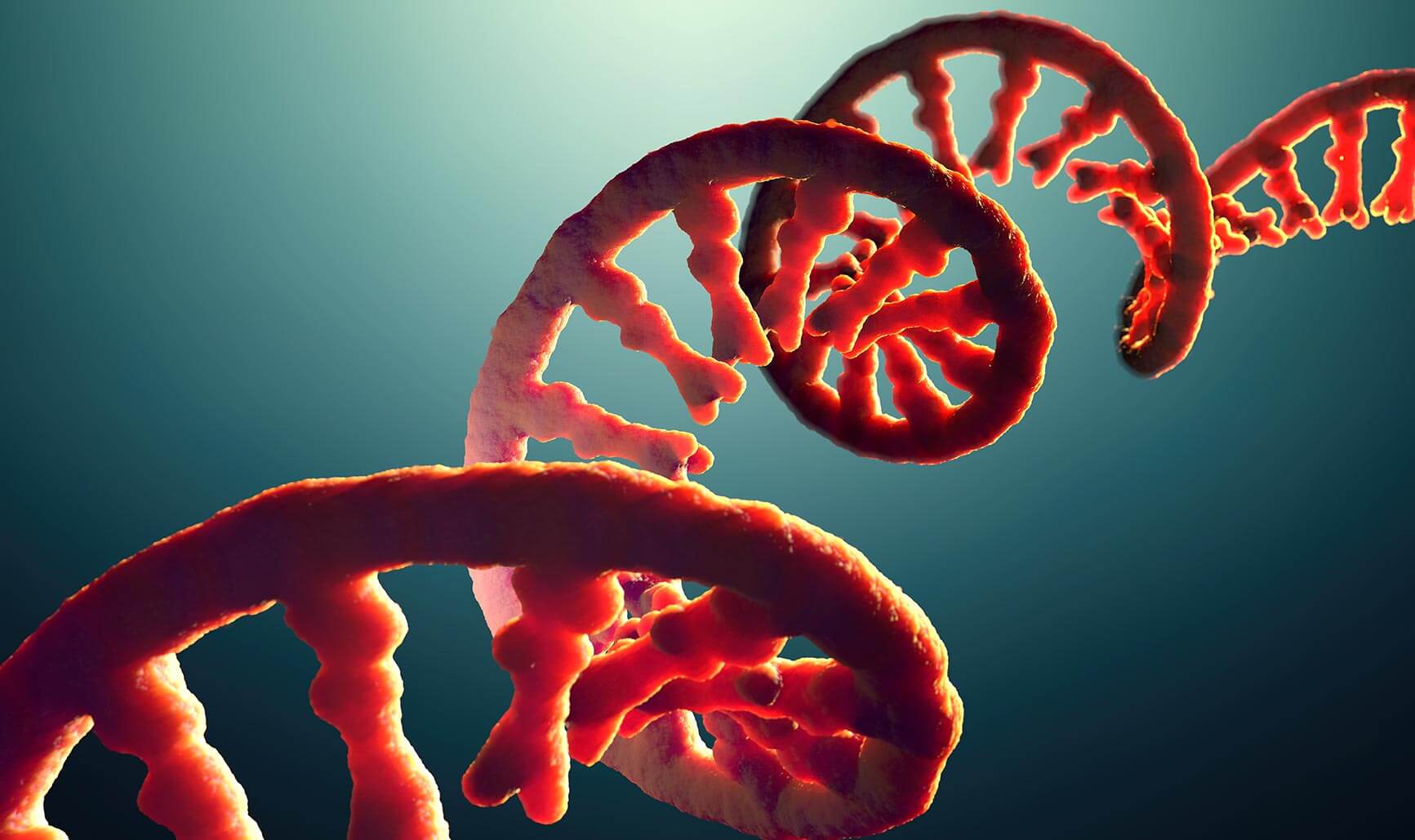
Welcome to the latest edition of our SoCal Biosciences newsletter. We’re glad to have you with us as we explore the fascinating world of mRNA detection. At SoCal Biosciences, we take pride in using the most advanced and reliable techniques to gain insights into mRNA distribution and expression. In this edition, we’ll walk you through some key mRNA detection methods, their development, applications, and why we emphasize using RT-PCR and bDNA assays in our work.
The Importance of mRNA Detection
mRNA detection plays a crucial role in both research and clinical applications. It helps us understand gene function, regulatory mechanisms, and how tissues respond to external stimuli or therapeutic interventions. Given the growing interest in mRNA-based therapies, particularly after the COVID-19 pandemic, accurate and reliable mRNA detection has become more important than ever.
In Situ Hybridization (ISH): A Historical Perspective and Technological Evolution
In Situ Hybridization (ISH) is a well-established technique in molecular biology that allows us to detect and localize specific nucleic acid sequences within tissue sections or whole cells. This method, which enables the visualization of mRNA within its natural cellular environment, has its roots in the pioneering work of Joseph Gall and Mary Lou Pardue in the 1960s. They first demonstrated the technique by detecting DNA in Drosophila chromosomes.
Gall and Pardue’s method involved using radiolabeled nucleic acid probes that hybridized to complementary DNA or RNA sequences within fixed tissues. The resulting hybridization signal, detected via autoradiography, provided a visual representation of the nucleic acid’s location within the tissue. This breakthrough was significant because it allowed scientists to study the distribution and organization of genes within chromosomes in a way that had not been possible before.
Over the years, ISH has evolved significantly. The introduction of non-radioactive labeling methods in the 1980s, such as digoxigenin or biotin-labeled probes, made ISH safer and more accessible. These probes can be detected using colorimetric or fluorescent methods, which not only improve safety but also enhance the resolution and ease of interpretation of results.
Fluorescence In Situ Hybridization (FISH) was a major milestone that emerged from these advancements. FISH uses fluorescently labeled probes to detect specific DNA or RNA sequences in tissues or cells, allowing for simultaneous visualization of multiple targets (multiplexing). This is particularly useful in medical diagnostics and research, where the spatial context of gene expression can provide insights into disease mechanisms or developmental biology.
Another significant development is Single Molecule FISH (smFISH), which further increases the sensitivity of ISH by enabling the detection of individual mRNA molecules. smFISH uses multiple probes that bind to different regions of the target mRNA, each labeled with a fluorescent dye. The cumulative signal from these probes allows for the precise localization and quantification of single mRNA molecules within cells. This technique has been revolutionary in studying gene expression at the single-cell level, providing insights into cellular heterogeneity and gene regulation that were previously inaccessible.
ISH, including its advanced forms like FISH and smFISH, remains a vital tool for studying gene expression patterns, diagnosing genetic disorders, and exploring the molecular architecture of tissues. As this technology continues to advance, we’re excited to see how it will further deepen our understanding of complex biological systems.
RNA Sequencing (RNA-Seq): Revolutionizing Transcriptomics
RNA Sequencing (RNA-Seq) has transformed the study of the transcriptome—the complete set of RNA transcripts produced by the genome. This powerful and versatile technique allows us to analyze gene expression across different conditions and time points, providing a comprehensive view of the dynamic nature of the transcriptome.
The development of RNA-Seq was a natural progression from earlier sequencing technologies. Introduced in the early 2000s, RNA-Seq quickly surpassed microarrays by offering higher sensitivity and the ability to detect novel transcripts. The first RNA-Seq experiments, published around 2008, marked a significant shift in how researchers studied gene expression. These early studies demonstrated the ability of RNA-Seq to detect both known and novel transcripts, quantify gene expression levels with high accuracy, and identify alternative splicing events and other post-transcriptional modifications.
Since its inception, RNA-Seq has undergone continuous refinement. Early versions of RNA-Seq were limited by the short read lengths and relatively high error rates of the sequencing platforms available at the time. However, as sequencing technologies improved, so too did the capabilities of RNA-Seq. One of the key advancements was the development of paired-end sequencing, which allowed for longer and more accurate reads, making it possible to assemble transcriptomes de novo and detect complex splicing patterns.
Another important development was the creation of specialized protocols for different types of RNA-Seq, such as single-cell RNA-Seq (scRNA-Seq). Single-cell RNA-Seq enables the analysis of gene expression at the level of individual cells, revealing cellular heterogeneity that would be obscured in bulk RNA-Seq data. This technique has provided profound insights into developmental biology, cancer, immunology, and more by uncovering the intricate gene expression landscapes within complex tissues.
Spatial Transcriptomics, a more recent innovation, integrates RNA-Seq with spatial information about where transcripts are located within tissues. This approach allows researchers to study gene expression in the context of tissue architecture, providing a deeper understanding of how gene expression patterns relate to tissue function and disease.
Today, RNA-Seq is a cornerstone of modern research, with applications ranging from identifying disease biomarkers to exploring the roles of non-coding RNAs. As this technology continues to evolve, it will undoubtedly lead to new discoveries and further our understanding of cellular function and disease.
Nanostring Technology and Luminex Assays: Precision Meets Multiplexing
Let’s move on to two incredible technologies that allow us to measure multiple targets simultaneously with high precision: Nanostring and Luminex. Nanostring Technology, particularly through its nCounter System, represents a significant advancement in the field of molecular diagnostics and gene expression analysis. The development of this technology was driven by the need for a robust, multiplexed method to directly measure nucleic acid molecules without the need for amplification, a common step in many other techniques like PCR.
The Nanostring nCounter platform was first introduced in the early 2000s by a company called NanoString Technologies, founded in 2003. The technology was built on the principle of digital molecular barcoding. Unlike traditional methods, which often require amplification of the target RNA or DNA, Nanostring’s system uses color-coded molecular barcodes that are hybridized directly to the target molecules. These barcodes are then digitally counted, providing a direct, quantifiable readout of gene expression levels.
The key advantage of Nanostring technology is its ability to measure multiple targets simultaneously with high precision, even from samples with low RNA quality or quantity. This makes it particularly valuable for analyzing clinical samples, such as formalin-fixed, paraffin-embedded (FFPE) tissues. The simplicity of the workflow and the lack of amplification steps reduce the potential for errors and biases that can affect other methods, such as PCR.
Nanostring has since expanded its technology to include additional applications, such as protein detection, miRNA profiling, and multiplexed analysis of gene expression in combination with spatial information. The flexibility and accuracy of this platform have made it a popular choice in both research and clinical settings, particularly for biomarker discovery and validation.
Similarly, Luminex Assays, developed by Luminex Corporation in the mid-1990s, revolutionized multiplexed detection by enabling the simultaneous analysis of up to 500 analytes in a single sample. The technology is based on the use of color-coded beads, known as microspheres, which are coated with specific capture antibodies or oligonucleotides. Each bead is assigned a unique fluorescent signature, allowing for the identification of the analyte it captures.
The development of Luminex technology was a response to the growing need for high-throughput, multiplexed assays in research and diagnostics. Traditional methods, such as ELISA (Enzyme-Linked Immunosorbent Assay), while effective, were limited by their capacity to measure only one analyte at a time. Luminex assays overcame this limitation by enabling the simultaneous quantification of up to 500 different analytes in a single reaction. This was achieved through the innovative use of flow cytometry, where beads are passed through a laser detector that reads the fluorescent signals of both the bead and the captured analyte.
Luminex assays have found widespread applications in various fields, including immunology, infectious disease research, and pharmacogenomics. In particular, the technology is extensively used for cytokine profiling, where the ability to measure multiple cytokines in a single sample is crucial for understanding the complex signaling networks involved in immune responses.
In recent years, Luminex technology has been adapted for use in gene expression analysis, similar to the applications seen with Nanostring. For instance, the xMAP Technology platform developed by Luminex allows for the simultaneous analysis of multiple RNA or DNA targets, providing a powerful tool for researchers studying complex gene expression patterns.
Both Nanostring Technology and Luminex Assays represent significant advancements in the field of multiplexed molecular detection. They address the limitations of earlier techniques by enabling the simultaneous analysis of multiple targets with high precision and minimal sample input. These technologies have become indispensable tools in both research and clinical diagnostics, driving forward our understanding of gene expression and its role in health and disease.
RT-PCR: The Gold Standard
No discussion of mRNA detection would be complete without mentioning RT-PCR, a method that has stood the test of time. Reverse Transcription-Polymerase Chain Reaction (RT-PCR) has long been a cornerstone of molecular biology. Developed in the late 1980s, RT-PCR combines reverse transcription of RNA into complementary DNA (cDNA) with PCR amplification, enabling precise measurement of specific RNA molecules. This technique quickly became a staple in molecular biology due to its sensitivity, specificity, and quantitative capabilities.
The origins of RT-PCR can be traced back to the invention of PCR by Kary Mullis in 1983, which revolutionized molecular biology by allowing the exponential amplification of DNA sequences. However, because PCR was limited to amplifying DNA, it couldn’t be used to directly analyze RNA, the molecule responsible for transmitting genetic information from DNA to the protein-synthesizing machinery of the cell. The breakthrough came with the realization that RNA could be reverse transcribed into cDNA, a stable DNA form that could then be amplified using PCR. This combination, first described in scientific literature in the late 1980s, led to the development of RT-PCR.
RT-PCR quickly gained popularity for its ability to quantitatively measure gene expression, enabling researchers to study how genes are regulated under different conditions and how gene expression patterns change in response to various stimuli. This made RT-PCR an invaluable tool in fields ranging from developmental biology to cancer research.
Over the years, RT-PCR has undergone several advancements, most notably the development of quantitative RT-PCR (qRT-PCR), also known as real-time PCR. This enhancement allows for the quantification of cDNA during the PCR process in real-time, rather than only at the end. qRT-PCR uses fluorescent dyes or probes that emit a signal proportional to the amount of DNA amplified, providing a quantitative measure of gene expression levels.
qRT-PCR represented a significant leap forward in molecular biology, as it combined the sensitivity and specificity of PCR with the ability to measure gene expression quantitatively. It has become a standard method in laboratories worldwide for applications such as validating microarray data, measuring the levels of gene expression, and detecting and quantifying pathogens like viruses.
In addition to qRT-PCR, several other variations of the technique have been developed, each tailored to specific research needs. For example, digital PCR (dPCR) offers even greater precision by partitioning the sample into thousands of individual reactions, allowing for the absolute quantification of nucleic acids without the need for standard curves. This has made dPCR particularly valuable in detecting low-abundance targets, such as rare mutations or minimal residual disease in cancer patients.
Today, RT-PCR is widely used in both research and clinical diagnostics. Its applications range from basic research, where it is used to study gene expression patterns, to clinical settings, where it is employed to detect and quantify RNA viruses, such as HIV and SARS-CoV-2. The COVID-19 pandemic, in particular, highlighted the critical role of RT-PCR in public health, as it became the gold standard for detecting SARS-CoV-2, the virus responsible for COVID-19.
The technique’s ability to provide rapid, accurate, and sensitive detection of RNA makes it indispensable in diagnostics, particularly for infectious diseases. Moreover, its quantitative capabilities are crucial for monitoring viral load in patients, guiding treatment decisions, and assessing the effectiveness of antiviral therapies.
As molecular biology continues to advance, RT-PCR remains a fundamental tool, continually evolving to meet new challenges in research and diagnostics. Its impact on science and medicine is profound, and it will undoubtedly continue to play a pivotal role in the study of gene expression and the detection of RNA-based pathogens.
Branched DNA (bDNA) Assay: Signal Amplification for Accurate Quantification
The Branched DNA (bDNA) assay is a signal amplification technique used for the quantification of nucleic acids, such as RNA and DNA, in various biological samples. Unlike traditional amplification methods like PCR, which amplify the target nucleic acid itself, the bDNA assay amplifies the signal generated from hybridization, making it a robust and reliable method for quantifying nucleic acids, particularly in clinical diagnostics and research settings.
The bDNA assay was developed in the early 1990s as an alternative to PCR-based techniques. It was introduced by Chiron Corporation (later acquired by Novartis) and became a key technology in the field of molecular diagnostics. The primary motivation behind the development of the bDNA assay was to create a method that could provide highly sensitive and specific quantification of nucleic acids without the need for the amplification of the target sequence itself, which can sometimes lead to biases or errors.
The bDNA assay leverages a unique approach to signal amplification that involves multiple layers of hybridization. The assay uses a series of synthetic oligonucleotide probes that are designed to hybridize to the target nucleic acid. These probes are attached to a branched DNA structure, which allows multiple signal amplification molecules to bind to a single target molecule. The branched structure increases the number of binding sites available for signal generation, leading to a significant amplification of the detection signal without increasing the amount of the target nucleic acid.
This method of signal amplification made the bDNA assay particularly valuable for applications where the sample quantity was limited, or where the target nucleic acid was present in very low abundance, such as in viral load monitoring in HIV patients. The technique was soon adopted for a range of diagnostic applications, including the quantification of viral RNA in clinical samples and the measurement of gene expression levels in research studies.
One of the most significant advantages of the bDNA assay is its ability to provide quantitative data across a wide dynamic range, with high reproducibility and minimal risk of contamination, which can be a concern in PCR-based methods. The assay’s reliance on signal amplification rather than target amplification means that it can accurately quantify nucleic acids in complex samples, such as those containing high levels of background RNA or DNA.
Over time, the bDNA assay has been refined and integrated into various automated platforms, making it easier to use in both research and clinical laboratories. Its application has expanded to include the quantification of mRNA, microRNA, and other small RNAs, as well as the detection of mutations and polymorphisms in genetic studies. The technology has also been adapted for use in multiplex formats, allowing for the simultaneous quantification of multiple targets in a single sample.
Today, the bDNA assay is widely used in clinical diagnostics, particularly for monitoring viral load in patients with chronic viral infections like HIV and hepatitis C. Its robustness and reliability have made it a gold standard in these applications, where accurate quantification is crucial for guiding treatment decisions.
In research, the bDNA assay is particularly valuable for gene expression analysis in studies requiring high sensitivity, especially when target nucleic acids are present at low levels. This technology’s ability to accurately quantify nucleic acids without extensive sample preparation or amplification steps makes it ideal for challenging sample types. For instance, the bDNA assay was employed to assess the biodistribution of mRNA-1647, a vaccine containing six different mRNAs of CMV origin, in Sprague-Dawley rats. This method allowed for the quantitative analysis of mRNA in various tissues without the need for RNA purification, enabling the detection of the vaccine’s presence in multiple organs, including the blood, injection site, lymph nodes, liver, spleen, and eyes.
As molecular diagnostics and personalized medicine continue to evolve, the bDNA assay is likely to remain a key technology, particularly as new applications emerge that require highly sensitive and specific nucleic acid quantification. Ongoing developments in probe design and signal detection may further enhance the assay’s performance, extending its utility in both clinical and research settings.
Why SoCal Biosciences Focuses on RT-PCR and bDNA
At SoCal Biosciences, we prioritize the use of RT-PCR and bDNA assays in our tissue distribution studies because of their superior sensitivity, specificity, and reliability. Both techniques offer robust, quantitative data essential for understanding mRNA distribution within tissues—a critical factor in the development of effective RNA-based therapies. While other methods like ISH, RNA-Seq, Nanostring, and Luminex have their strengths, RT-PCR and bDNA stand out for their precision and utility in complex biological samples.
As you continue to advance your research and development of new therapeutic solutions, we remain committed to supporting you using the most effective and reliable technologies available. RT-PCR and bDNA assays will continue to be central to our work, ensuring that your results are both accurate and impactful.
For a deeper dive into these techniques, we encourage you to explore the publications listed below.
Thank you for being a part of our journey in advancing mRNA detection. We look forward to sharing more insights and discoveries with you in future editions.
Publications
- Ramos JM. Fluorescent In Situ Hybridization (FISH). Methods Mol Biol. 2022;2422:179-189. doi: 10.1007/978-1-0716-1948-3_12. PMID: 34859406
- Tsanov N, Samacoits A, Chouaib R, Traboulsi AM, Gostan T, Weber C, Zimmer C, Zibara K, Walter T, Peter M, Bertrand E, Mueller F. smiFISH and FISH-quant – a flexible single RNA detection approach with super-resolution capability. Nucleic Acids Res. 2016 Dec 15;44(22):e165. doi: 10.1093/nar/gkw784. Epub 2016 Sep 5. PMID: 27599845; PMCID: PMC5159540.
- Wang Z, Gerstein M, Snyder M. RNA-Seq: a revolutionary tool for transcriptomics. Nat Rev Genet. 2009 Jan;10(1):57-63. doi: 10.1038/nrg2484. PMID: 19015660; PMCID: PMC2949280.
- Conesa A, Madrigal P, Tarazona S, Gomez-Cabrero D, Cervera A, McPherson A, Szcześniak MW, Gaffney DJ, Elo LL, Zhang X, Mortazavi A. A survey of best practices for RNA-seq data analysis. Genome Biol. 2016 Jan 26;17:13. doi: 10.1186/s13059-016-0881-8. Erratum in: Genome Biol. 2016 Aug 26;17(1):181. doi: 10.1186/s13059-016-1047-4. PMID: 26813401; PMCID: PMC4728800.
- Goytain A, Ng T. NanoString nCounter Technology: High-Throughput RNA Validation. Methods Mol Biol. 2020;2079:125-139. doi: 10.1007/978-1-4939-9904-0_10. PMID: 31728967.
- Tsang HF, Xue VW, Koh SP, Chiu YM, Ng LP, Wong SC. NanoString, a novel digital color-coded barcode technology: current and future applications in molecular diagnostics. Expert Rev Mol Diagn. 2017 Jan;17(1):95-103. doi: 10.1080/14737159.2017.1268533. Epub 2016 Dec 12. PMID: 27917695.
- Dunbar SA. Applications of Luminex xMAP technology for rapid, high-throughput multiplexed nucleic acid detection. Clin Chim Acta. 2006 Jan;363(1-2):71-82. doi: 10.1016/j.cccn.2005.06.023. Epub 2005 Aug 15. PMID: 16102740; PMCID: PMC7124242.
- Wong ML, Medrano JF. Real-time PCR for mRNA quantitation. Biotechniques. 2005 Jul;39(1):75-85. doi: 10.2144/05391RV01. PMID: 16060372.
- Nouwairi RL, Cunha LL, Turiello R, Scott O, Hickey J, Thomson S, Knowles S, Chapman JD, Landers JP. Ultra-rapid real-time microfluidic RT-PCR instrument for nucleic acid analysis. Lab Chip. 2022 Sep 13;22(18):3424-3435. doi: 10.1039/d2lc00495j. PMID: 35959772; PMCID: PMC9474628.
- Pateev I, Seregina K, Ivanov R, Reshetnikov V. Biodistribution of RNA Vaccines and of Their Products: Evidence from Human and Animal Studies. Biomedicines. 2023 Dec 26;12(1):59. doi: 10.3390/biomedicines12010059. PMID: 38255166; PMCID: PMC10812935.
We hope you enjoyed this deep dive into RNA detection techniques. But we’re not stopping here! In our next edition, we’ll be shifting gears and focusing on protein detection methods—another crucial area of research and development. Get ready for some exciting insights and cutting-edge advancements!
Join leading researchers and drug developers who trust our bioanalytical CRO expertise. Receive exclusive content, industry insights, and innovative methodologies straight to your inbox.